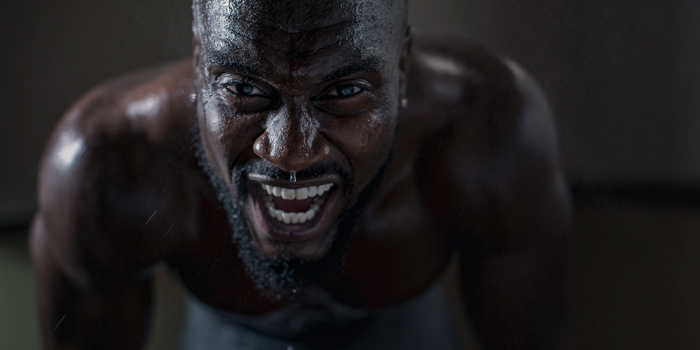
In order to delineate the process of fatigue, it is important to ask the question as to why fatigue is coming about in the first place. Why would the body want to inhibit its contractile components and reduce its ability to protect itself (say, if you’re needing to run from a lion)? As will be seen, this is not a random or illogical occurrence.
Breaking it down to the basic processes, the body receives a stimulus, determines what it should do, and then responds to this stimulus. The sensory response can be transmitted to the central nervous system via a myriad of sensory receptors. This information is then integrated into the central nervous system and a subsequent response is initiated. Many areas within this sensory-motor system can be negatively affected by the fatiguing process. As this system continues (as with exercise), various changes arise, at both the neural and muscular level, which is constantly being monitored by the higher command centers (the brain).
Taking this perspective with exercise, it seems rather obvious that fatigue is an inevitable response to a training session. Fatigue, though, is a relative term, and thus must be defined. As defined by Digby Sale, fatigue is the failure to continue exercising at a set intensity. Failure to activate a muscle, however, is not the type of fatigue we will be looking at. As described by Gandevia, “The key problem is that multiple processes and mechanisms are involved at different levels, from higher-order CNS structures to electrical and biochemical alterations within muscle fibers”. This being the case, the goal of this article is to look at these neural and biochemical changes during the fatiguing process rather than the end effect.
RELATED: Sleep More, Lift More
The fatiguing process is continuous and begins at the onset of exercise, regardless of the intensity and/or duration. Expounding upon the divergent pathways that are underway dependent upon the intensity and/or duration, Sale and MacDougall describe that:
“In the brain, there could be decreased volitional drive to the motor cortex. The motor cortex would then decrease excitation of the motoneurons which would result in reduced MU recruitment and decreased MU firing rates. In addition, the motoneurons might become less excitable, further increasing the possibility of MU drop-out and decreased firing rates. There could also be reflex inhibition or reduced reflex excitation that would also act to cause MU drop-out and decreased MU firing rates. There may be neuromuscular transmission failure that would cause muscle fiber drop-out. Excitation-contraction coupling might be impaired, which would decrease the force of contraction. Finally, there are metabolic factors such as fuel depletion and metabolite accumulation that have been implicated in fatigue, perhaps having a direct effect on the level of cross-bridges and an indirect effect such as reducing the number of active cross-bridges by, for example, reflex inhibition and impaired excitation-contraction coupling.”
Each of these areas described by Sale and MacDougall will be touched upon in this article.
Cellular Mechanisms and Fatigue
Taking a look at the singular muscle fiber, it is apparent that for any type of muscular activity to be initiated, many different chemical reactions must take place. With these various chemical reactions taking place, metabolites are subsequently accumulated. With inadequate recovery time and/or inadequate oxidative properties for the set exercise scheme, these accumulated metabolites will inhibit the many aspects of the excitation-contraction pathway. The metabolites we'll discuss are inorganic phosphate, magnesium, lactate, and hydrogen ions.
Inorganic Phosphate
Inorganic phosphate (Pi) has caused many exercise physiologists countless headaches in relation to its role in the fatiguing process. Once lactate accumulation was ruled out (which it soon will be), inorganic phosphate accumulation looked like the likely culprit.
The rise of inorganic phosphate is primarily due to the hydrolysis of creatine phosphate during intense bouts of exercise. While it has been well established that increased Pi levels decrease the sensitivity of calcium to the myofilaments within the muscle fiber, along with decreasing the activity of the sarcoplasmic reticulum, subsequently reducing the calcium quantity in the myoplasm and thus a decrease in the muscular contractility, other studies have questioned this and gives credence to a brief review of this specific area.
Concerning Pi in the failure of the sarcoplasmic reticulum (SR) calcium release during fatigue (remember, calcium is stored in the sarcoplasmic reticulum), it has been demonstrated that Pi, when increased in intracellular quantity, causes slowing of the SR pump, which reduces the quantity of Ca2+ released, along with its decrease of calcium taken back up. While prevalent in relation to reduced SR calcium release, it appears to be only one of many possible factors of which Pi plays a role.
During fatiguing exercise, inorganic phosphate can make its way into the sarcoplasmic reticulum via anion channels. When a large amount of Pi is taken up, as described by Fryer, this metabolite will bind with calcium and form calcium phosphate. The binding affinity of calcium phosphate is strong and this degrades the level of free calcium to be pumped out of the sarcoplasmic reticulum during times of muscular contraction. It is speculated that this is the primary cause of tetanic calcium reduction.
Taking this into consideration, Fryer and colleagues demonstrated a linear relationship with inorganic phosphate accumulation and reduction of the maximal tension generated from the specific muscle fiber. This reduction in maximal tension with an increase in inorganic phosphate, along with a decrease in calcium sensitivity, while noticeable in all fiber types, appears to be of greater importance with slow twitch fibers as compared to fast twitch fibers, which, when tested, required greater Pi concentration to reduce calcium sensitivity.
Thus, the impact inorganic phosphate has on the specific type of fatigue is highly dependent upon the myofiber’s phenotypic characteristics. Regardless, we can see that increased Pi can cause an issue by:
- Decreased activity of the sarcoplasmic reticulum pumps (inhibited ATP-driven SR Ca2+ uptake)
- Decreased level of free calcium in the myoplasm via formation of calcium-phosphate
- Decreased myofibrillar calcium binding sites due to Pi interference
Magnesium
Increased intracellular magnesium (Mg2+) has also shown to inhibit muscle fiber contractile properties predominately due to an increased quantity of adenosine diphosphate (ADP), adenosine monophosphate (AMP), and inosine monophosphate (IMP). This increase in ADP, AMP, and IMP occur due to the relatively slow resynthesis of ATP compared to its breakdown and utilization (this greatly depends upon one’s oxidative enzymatic properties and exercise intensity/duration). These three products have a decreased affinity of magnesium than does ATP. Thus, the reduction of the ATP:ADP/AMP/IMP ratio subsequently causes an indirect increase in Mg2+.
The predominant effect of an increased concentration of Mg2+, similar to the other accumulated metabolites, is a decrease in calcium (Ca2+) sensitivity. Furthermore, it was demonstrated that increased Mg2+ levels inhibited Ca2+ efflux via inhibiting the sarcoplasmic reticulum release channels along with reducing the voltage sensing properties, which also reduces Ca2+ release. Again, the primary cause of this is a decrease in ATP as compared to the increase in ADP, AMP, and IMP and subsequent increased Mg2+ concentration.
Hydrogen Ions, pH, and Lactate
Another key metabolite that has been expounded upon concerning fatigue at the cellular level are hydrogen ions. Hydrogen ions seem to get a bad rap due to their ability to decrease the intracellular pH. It has been shown multiple times, however, that an individual’s pH does not directly correlate with force producing qualities. On the contrary, some have reached a state of fatigue with a pH of 6.9 (close to normal) whereas others have been as low as 6.5. Similarly, it was shown that, following an exhaustive bout of exercise, force output increased faster than the pH when returning to baseline. This being the case, it is apparent that H+ accumulation and pH reduction may not be a leading cause of the fatiguing process.
Now, taking the above into consideration, this by no means releases H+ and an acidic environment from some of the negative effects on the muscle fibers contractile properties. An increased acidic environment and subsequent decrease in pH does cause an issue with calcium sensitivity. Specifically, H+ accumulation has been shown to compete with calcium binding to troponin.
Looking at the sliding filament theory, this would then reduce the number of actin binding sites and inevitably lead to decreased muscle activity. However, an interesting twist is, while the troponin decreases its affinity in relation to Ca2+, the sarcoplasmic reticulum pump also reduces its affinity of Ca2+, which allows for a greater cytoplasmic free Ca2+ content, which then could lead to increased binding onto troponin and subsequent tropomyosin shift.
While this may be true, if we recall the effect that an increased inorganic phosphate has on sarcoplasmic reticulum Ca2+ quantity, it would seem clear that free Ca2+ would still be decreased, albeit, not due to a decrease in pH.
Furthermore, Edman demonstrated that a reduced pH has been shown to “reduce the rate of rise of force during the onset of tetanus and slowing of the linear phase of force relaxation.” Similarly, Edman showed that a reduced intracellular pH, such as that found during fatigue, increases the fiber's ability to resist stretch, which is thought to come about by making the myosin cross-bridges attach more firmly to the actin filaments during activity.
Summing up H+ and pH during fatigue, it appears that an increased H+ intracellular level, while still somewhat speculative, is not a primary cause of fatigue but may cause an issue with calcium kinetics. Similarly, it appears that a reduced pH can cause an issue with myofiber activity. This is still being looked at due to the disagreement in findings.
This now leads to lactate. I will not talk much about lactate's role in fatigue, simply because it has been accepted for decades that lactate is not a dead-end waste product and does not play a significant role in the fatigue phenomenon. Rather, lactate helps mitigate the onset of fatigue via the buffering of protons and increases available glucose by means of gluconeogenesis.
Furthermore, lactate plays a large role in wound repair, along with being the primary fuel source for neurons within the brain — not to mention lactates role as a signaling molecule upregulating over 600 genes, predominately those that deal with mitochondrial biogenesis. Suffice it to say, lactate production is beneficial and should not be thought of as a wasteful metabolite.
Cell Swelling and Reactive Oxygen Species
It has often been thought that metabolite accumulation, such as inorganic phosphate, magnesium, lactate, and hydrogen ions, cause an increase in cell swelling which, if true, has shown to cause reduction in skeletal muscle contractile properties. Looking at the work of Usher-Smith and colleagues, they describe that:
“It is apparent that Lac(tate) production reduces the magnitudes of the cellular shrinkage and intracellular acidification that result from H+ production, while H+ production offsets the swelling of Lac(tate) production and produces an acidification that favors lactate efflux, and thus each ion ameliorates the effects upon Vc (cell volume) of the high rates of production of the other that occur in exhaustive anaerobic exercise.”
This is incredibly interesting because it shows that metabolite accumulation, other than lactate (in this instance hydrogen ions), plays a very important role in maintaining cellular homeostasis, and is not simply the culprit. In this case, this role is mitigating cell swelling.
Having said this, the volume of a fatigued muscle fiber has been shown to be 180% greater in size than of a muscle fiber at rest. A possible mechanism of cellular swelling could be due to the natural occlusion of contractile muscles, specifically during intense bouts of activity. This cellular swelling has been shown to impact the resting membrane potential due to the mechanosensitive properties of the K+ channels, causing an issue with the neural mechanisms of the muscle fiber and subsequent activation of the sarcoplasmic reticulum.
Now looking at reactive oxygen species (ROS) role in fatigue, ROS activity and production increases with muscular activity. When looking at the cause of ROS production during intense activity, it has been shown that the mitochondria oxygen consumption produces superoxide (a type of ROS), which then diffuses across the mitochondrial membrane into the myoplasm via its conversion into hydrogen peroxide (H2O2). Other mechanisms of mitochondrial ROS production have also been shown which is increased when oxygen demands are high such as intense exercise.
Similar to hydrogen ion and inorganic phosphate accumulation, increase in ROS activity seems to negatively impact the calcium kinetics of the working muscle. This can be through a reduction in calcium sensitivity, the reduction of calcium release from the sarcoplasmic reticulum (although not probable), and a reduced uptake of calcium into the sarcoplasmic reticulum. Furthermore, increased levels of ROS have been shown to breakdown both troponin I and C, thus causing an issue with the contractile components of the muscle fiber.
Metabolic Feedback Mechanisms
Looking at the feedback mechanisms, it is rather straightforward as to the process and reasoning behind a feedback response causing reduced efferent signaling to the given muscle fiber. If we break it down to its basic components, we can see that the primary cause of this feedback mechanism arises from various pathways signaling that the ATP equilibrium is below what is necessary for the activity taking place. As described by Noakes:
“Multiple, independent systems in the periphery provide sensory feedback that influences central motor drive from the brain to the exercising muscles.”
An example of this, as previously mentioned, is an increase in ADP, AMP, or IMP, which signifies an increased ATP hydrolysis as compared to resynthesis. These feedback chemicals are then apparent to have been produced via ATP utilization, such as Pi, ADP, H+, and Creatine.
This is, once a given metabolite threshold is met, presented as a threat to the brain, causes subsequently decreased activation of the specific motor unit. A lot of this is due to inter-neuronal communication via chemo- and mechanoreceptors leading to its interpretation and decreased motoneuron discharge.
Neural Feedback Mechanisms
Continuing, looking at the neural feedback mechanisms, it has been postulated that fatigue can be brought about by reduced muscle spindle activity, the golgi tendon apparatus, and various mechanoreceptors dependent upon location.
Concerning muscle spindle activity under fatiguing bouts, adequate function is necessary for proper sensory input, integration in the central nervous system, and subsequent motor response. This reduction in muscle spindle activity has been proposed to be due to altered fusimotor drive to the spindles. This then can lead to a decreased afferent firing rate. This feedback mechanism in multifaceted and is due to a number of factors of varying degrees. The end result, however, focuses around motor unit firing alteration.
The golgi tendon organ (GTO), while not as well understood as the muscle spindle in relation to fatigue, still plays a role in transmitting information to the motor transmitting apparatus. Specifically, when looking at the Ib afferent discharge due to the GTO activation, this specific feedback mechanism can cause an inhibitory signal and subsequently altered motor response. These Ib afferents have also been shown to be affected by pre-synaptic inhibition leading to a further reduction in motoneuron activity.
As previously mentioned, due to altered sensory information in states of fatigue, motor units tend to change their function. Two of the predominant ways that this takes place is through frequency optimization (the rate at which it fires) and frequency patterning (the variability within the firing discharge). Concerning frequency optimization, during sustained maximal contraction, the discharge has been shown to decrease. Along with this, looking at frequency patterning, the time-course of contraction-relaxation of the entire muscle (including motor units) change which then can further change the timing of motor unit activity.
This section has been focused on the feedback information of the peripheral musculature, causing changes in motor discharge activity during fatigue. However, as will soon be described, the "command center" (brain) can also dictate motoneuron activity as a precaution mechanism. This term is considered the Central Governor Model.
Central Governor Model
The factors put forth so far can most certainly shed light on some of the effects of fatigue. However, when looking at total muscle activation, it is apparent that many individuals frequently tire prior to full motor unit recruitment. This then must lead one to conclude that there was some reduced neural component during the exercising process.
This is thought to deal with an anticipatory response put forth by the brain as a means to protect the muscular system, among the many organs of the body, from any possible irreversible damage. This concept is widely proclaimed by the exercise scientist, Tim Noakes. However, Dr. Noakes was not the first to discuss this phenomenon. The revered Dr. A.V. Hill wrote about an anticipatory response of the heart during increasingly strenuous bouts of exercise. Having said that, Dr. Noakes is the primary researcher of the central governor model and will thus be the primary resource for this section.
In contrast to the feedback mechanism discussed above, the central governor model is a feedforward mechanism. The goal of the brain is to regulate homeostasis and, when given certain information about the environment of the body (feedback), it will change neural drive to the working musculature along with other organs (feedforward).
This model proposes that there is a continuous fluctuation and alteration of motor unit activation in response to internal and external stimuli. This has been shown repeatedly by Noakes and colleagues. In one interesting case, during an endurance activity, individuals were shown to alter their output abilities as recorded by EMG to different gas mixtures inspired with oxygen unbeknownst to the test-subject.
Another experiment which showed the prevalence of the Central Governor Model was conducted by the Red Bull Human Performance Laboratory. In this study, they looked at brain activity during intensive bouts of exercise with and without transcranial direct current stimulation. Transcranial direct current stimulation is speculated to modulate motor cortex activity and possibly override the inhibiting effect of the brain allowing for a greater neural drive to the working muscles. The results are currently preliminary with relatively few test-subjects. However, the results look promising.
LISTEN: Introducing the New Peak Mental Performance Podcast with Guest Dr. Steve Graef
The proponents of the Central Governor Model frequently raise issue, deservedly, with the peripheral fatigue model. This would appear to be due to the dogmatic and absolutist attitude toward the cause of fatigue. It is the author’s opinion that there need not be a separation of the central governor model and the peripheral fatigue model. It is clear that both play a role and are continuously present.
For example, can ADP/AMP/IMP accumulation lead to sensory feedback, causing a change in motor activity as proclaimed by the central governor model? Yes. Can ADP/AMP/IMP accumulation lead to increased Mg2+ concentration and subsequent issue with the contractile properties of the muscle fiber and thus cause immediate peripheral fatigue? Yes. A harmonious integration of the many processes within the human organism is necessary for the homeostasis-seeking body to successfully respond to the onset of fatigue.
Fatigue Individuality
Taking into consideration all of what has been described so far, inter-individuality in relation to fatigue must be given attention. The musculoskeletal, neuroanatomical, and cardiovascular systems are incredibly different in relation to hereditary and environmental stimuli. We know that there can be a wide range of responses to the same stimuli, which has been coined high and low responders.
Taking a closer look at this in regards to fatigue, the biochemical individuality plays an incredibly large role in the response to a specific type of fatiguing bout, and these variations can be magnified simply due to diurnal, lunar, and seasonal effects. For example, the enzymatic processes within the body which, along with other chemical reactions taking place, greatly differ between individuals independent of training history and current fitness state.
These differences have been shown to be as great as 10 to 50-fold in what is considered “normal” individuals. Some key areas looked at are within the oxidative process; specifically, looking at the ability to rid the intracellular environment of various accumulated metabolites.
The key point here is that, when prescribing any form of training for yourself or for your athletes, remember that they are unique individuals and will respond to training differently. If your athlete says he/she is tired while another athlete looks as fresh as can be, it would be unwise to say that specific athlete needs more “conditioning” while the other does not. The fatiguing processes are just as individual as the adaptation process.
Conclusion
This article by no means encapsulates the fatiguing process in its entirety. There are many other aspects of the body that are constantly being monitored, such as fuel reserves, one’s hydration state, body temperature, etc., which take part in the feedback-loop and subsequently affect the feed-forward response. What this article does offer is insight into some of the key players and lets the reader realize that, while some periods of "fatigue" may simply be in the individual's head, there are also processes taking place within the body which, due to its number one goal of survival, will cause issue with any further physical performance.
References
- Allen D. G., Lamb G. D., Westerblad H. (2008). Skeletal muscle fatigue: cellular mechanisms. Physiol. Rev. 88, 287–332. 10.1152/physrev.00015.2007
- Enoka R. M., Duchateau J. (2008). Muscle fatigue: what, why and how it influences muscle function. J. Physiol. 586, 11–23. 10.1113/jphysiol.2007.139477
- Gandevia S.C., Enoka R.M., McComas A.J., Stuart D.G., Thomas C.K. Fatigue: Neural and Muscular Mechanisms
- Noakes T.D. Time to move beyond a brainless exercise physiology: the evidence for complex regulation of human exercise performance. Appl. Physiol. Nutr. Metab. 2011;36(1):23–35. doi: 10.1139/H10-082
- Sale D, MacDougall D. (2014). The Physiology Of Training For High Performance.
- Usher-Smith JA, Fraser JA, Bailey PS, Griffin JL, Huang CL. The influence of intracellular lactate and H+ on cell volume in amphibian skeletal muscle. J Physiol. 2006;573:799–818.
- Westerblad H., Allen D.G., Lännergren J. Muscle fatigue: Lactic acid or inorganic phosphate the major cause? News Physiol. Sci. 2002;17:17–21
Luke Olsen is a current graduate student within the Exercise Physiology department at the University of Kansas with research interests related to exercise’s role in cellular and molecular physiology.